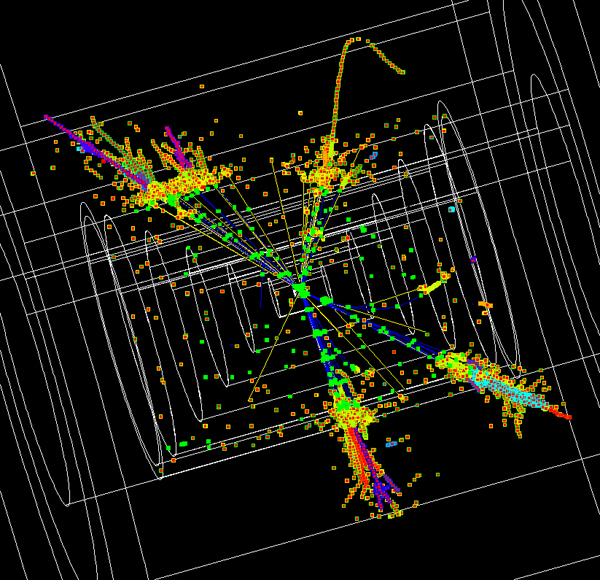
The Large Hadron Collider (LHC) is currently undergoing upgrades that will allow it to finally reach its intended top energy of 14TeV. When it comes back online, researchers will use it to probe the properties of the Higgs boson it discovered and to continue the search for particles beyond those described by the Standard Model. But no matter how many Higgs particles pop out of the machine, there's a limit to how much we can discover there.
That's because the hadrons it uses create messy collisions that are hard to characterize. The solution to this is to switch to leptons, a class of particles that includes the familiar electron. Leptons present their own challenges but allow for clean collisions at precise energies, allowing the machine to produce little beyond the intended particles. So now, the international physics community is putting agreements in place that will see a new lepton collider start construction before the decade is out, most likely in Japan.
Hadrons vs. leptons
Hadrons like the proton are composed of a mixture of quarks, gluons, and virtual particles. Their heavy mass makes them easy to accelerate. When fast-moving particles move around curves, some of their energy is lost as radiation; the proportion of lost energy goes down as the particle's mass goes up. So when lightweight electrons were sent through the curved tunnels that now house the LHC, the maximum energy they could reach was just over 100GeV. In contrast, protons can go through those same curves at 7TeV, meaning the collisions have significantly more energy.
The problem with hadrons comes from the fact that they're made up of a bunch of individual particles, and there's no way of controlling how the particles are oriented when the collision takes place. Even when two protons smash head-on, the quarks inside them may end up colliding off-center. As a result, only a small fraction of the total energy ends up being available to create new particles. Since there's no way of predicting how much, reconstructing what happened during a collision gets rather complicated, especially since each collision is really multiple smaller collisions. Imagine trying to reconstruct what happened when two trucks used to ship cars collide when you don't know which cars started on what truck.
Leptons, in contrast, are fundamental particles; there's nothing inside them, so when they collide, all the energy gets put into a single, simple collision. This means you can see things like the Higgs without accelerating the electrons to anywhere near the energies used at the LHC. It's also relatively easy to collide electrons with their antimatter cousins, the positrons, which eliminates the particles themselves in the process. The end result is a very clean collision and, if it's at the appropriate energy (say, near the mass of a heavy particle), a strong likelihood of producing the particle you're interested in.
Since leptons don't like running in circles, the solution is to build a linear collider. If everything moves in a straight line, it's possible to get electrons up to high energies and keep them there. The problem is that a straight line means long tunnels and infrastructure spread along them. All of which means a large expense.
ILC vs. CLiC
Even while the LHC was still being built, scientists started planning for a next-generation linear collider under the assumption that the LHC would spot the Higgs and physicists would want to study its properties in detail. Two competing camps were formed, one focused at Fermilab, the other at CERN.
The CERN group called its effort the Compact Linear Collider, or CLiC. To keep costs under control, CLiC would accelerate leptons within a shorter distance by transferring energy from one beam of electrons to the beams of electrons and positrons used for collisions. The result is a shorter (or, as the name implies, more compact) collider. The downside is that the technology remains unproven, so we don't know whether it would actually work in practice.
That dilemma left the focus on the International Linear Collider, which uses extensions of existing technology on a larger scale—a much larger scale. The initial plan for the ILC calls for the hardware to be housed in a tunnel 30km long; to increase the energy later, another 20km may be added. Initial plans were to build it at Fermilab, although the size meant that the tunnel would extend out under some of the neighboring towns. But the US Congress cut the funding for it a few years back, leaving the project in a bit of a limbo. Two factors have changed that: the actual discovery of the Higgs, which gives the collider its purpose, and the Great East Japan Earthquake of 2011, which freed up money from the Japanese government as part of the country's recovery efforts.
(It's worth noting that Japan hasn't been designated the official site yet, but that is an offer that will be hard to refuse. Also worth noting is that the ILC and CLiC teams are partly competitors and partly collaborators.)

The design calls for two storage rings, one each for the electrons and positrons. Electrons are relatively easy to come by, but the positrons will be made on-site. Basically, the electron beam will be sent on a curved path and lose energy via the production of high-energy photons. These photons will be converted to electron/positron pairs; the electrons will be discarded, and the positrons will be sent into the storage ring. From the ring, the electrons and positrons will be sent 15km in opposite directions, sent around a curve and then fed into two linear accelerators that are 11km long each and pointed roughly at each other.
If future higher-energy extensions go forward, they would basically involve extending the tunnels and shifting the point where the electrons go around a bend further out. More accelerator hardware would be attached to the backend of the existing linear accelerator, lengthening it so that the electrons spend more time being accelerated and come out at a higher energy.
There's a single beam-collision point at the center of the complex, which means only a single detector can take data from collisions. To provide the reassurance of reproducibility, there will be two detectors on rails, allowing one to be slid out and a second to be slid in to replace it. If there's enough shielding, it should be possible to perform maintenance on one while the second is operating.
Since the amount of acceleration can be tuned, the initial range of the accelerator runs from about 200GeV to 500GeV, enough to explore the production of the Higgs with a Z boson and see how the two interact (given that the Z is a relatively massive particle, the answer is expected to be "extensively"). At the high end, a pair of Higgs particles can be produced with a Z, allowing us to determine how the particle interacts with itself. Combinations of the Higgs and two top quarks are also possible (the top is the most massive particle we know about).
Extending the energy up to 1TeV would get us more elaborate combinations, but it's hard to see that much money being spent unless the LHC produces some evidence for supersymmetry, additional Higgs particles, or dark matter particles (or some combination of the above).
The LHC has plenty of time to get there, as a final site for the ILC hasn't even been chosen yet. It will take a few years to finalize the design specs and then roughly a decade to construct it, so operation probably won't start until the late 2020s. By that time, the LHC will probably have gone through a second round of upgrades and should have thoroughly explored all the particles accessible to the energies it can reach.
Source:http://arstechnica.com
No comments:
Post a Comment